ABSTRACT
This review article aims at giving insight into cellulose-based nanomaterials and their potential use for wound dressing applications. The first part will focus on nanocellulose particles, including their production, properties and assembly into highly organized materials. The design of 2D (films and nanopapers) and 3D structures (cryogels and aerogels) will also be discussed, and the application of nanocellulose-based materials in the biomedical field will be presented.
The benefits of using nanocellulose-based materials for wound-dressing applications will be considered and discussed in the second part. First, an introduction to microbiology and microorganisms encountered in wound infections will be presented. Then, bio-based wound dressings and related requirements will be assessed. Finally, the elaboration and characterization techniques of bioactive materials will be described. Nanocellulose functionalization will be discussed in the last section. The range of nanocellulose modification in green and traditional solvents will be extended to newer and innovative solutions for “green” functionalization with apolar solvents, with a special focus on the use of supercritical carbon dioxide.
Cellulose is a linear homo-polysaccharide of D-anhydroglucopyranose units (AGU) linked together by an equatorial glycosidic β-(1-4) linkage around which the AGUs are oriented at 180° from each other (Haworth 1930). Its molecular structure is illustrated in Figure 1a. Cellulose is the main component of plant cell walls and one of the basic structural units of algae, tunicates, and certain bacteria. The basic molecular formula of cellulose, C6H10O5, was established by Willstätter in 1913 (Willstätter and Zechmeister 1913), seventy-five years after its isolation from plant cell walls by Anselme Payen in 1838 (Payen 1838). The degree of polymerization, DP, is commonly expressed as the number of AGUs. The DP is usually a mean value since a wide distribution of chain length can be observed within the same sample. Cellulose has an average DP ranging between 1000 and 50000 depending on the source (e.g. 1500 for wood pulp cellulose, 20000 for secondary wall cotton cellulose and 50000 for Valonia algae). Each anhydroglucose unit has three hydroxyl groups : one primary alcohol on carbon 6 and two secondary alcohols on carbons 2 and 3. The two ends of one cellulose chain are not chemically equivalent. The so-called non-reducing end consists of a D-glucopyranose whose anomeric carbon is engaged in a glycosidic bond, and that has a free secondary alcohol function in position 4. The other end, referred to as the reducing end, is a D-glucopyranose unit whose anomeric carbon is free resulting in an equilibrium between a cyclic form and a minority open aldehyde form. This difference gives the cellulose chain a chemical polarity. Such a chemical asymmetry arises from the biosynthesis process.
Cellulose is largely biosynthesized in plants. Its synthesis and regulation have been widely studied and reported in the literature (Saxena and Brown 2005 ; Li et al. 2014). The glycan chains are assembled into microfibrils through van der Waals forces and both intra- and inter-molecular hydrogen bonds (Figure 1b). These numerous interactions, together with an amphiphilic behaviour, are considered to prevent the dissolution of cellulose in most solvents, as discussed by Lindman et al. (2010) and result in the formation of highly organized crystalline structures. Since the 1930s, numerous studies have been carried out to elucidate the crystal structure of cellulose (Honjo 1958 ; Meyer 1937 ; Sarko 1974). It is now known that cellulose can exist in the form of seven well-described allomorphs : Iα, Iβ, II, IIII, IIIII, IVI and IVII with different possibilities of conversion between them through chemical or thermal treatments (Figure I.4) (Chanzy 1979 ; Helbert 1997 ; Isogai 1994).
Native cellulose is type I. 13C solid-state NMR (Atalla 1984 ; Vanderhart 1984), and electron diffraction experiments (Sugiyama 1991) have shown that type I cellulose is in fact divided into two crystalline forms : a triclinic Iα phase with one chain per mesh and a monoclinic Iβ phase with two chains per mesh. The proportion of cellulose Iα and Iβ differs depending on the origin of the cellulose. Cellulose Iα is mainly found in primitive organisms such as in the wall of certain algae or produced by bacteria, whereas the Iβ phase predominates in plants such as cotton or the walls of tunicates (Belton 1989 ; Kono 2002).
Cellulose fibres play a major role in mechanical resistance in plant cell walls, where they are interacting with other biopolymers such as hemicelluloses, pectins, and lignins. The amount of cellulose, its degree of polymerization, crystallinity, orientation and its organization in the cell wall polymers complex is regulated during cellulose synthesis. Other organisms synthesize cellulose, among one which can cite tunicate (a sea animal), bacteria and algae (Kimura and Itoh 1996 ; Okuda et al. 2004 ; Esa et al. 2014).
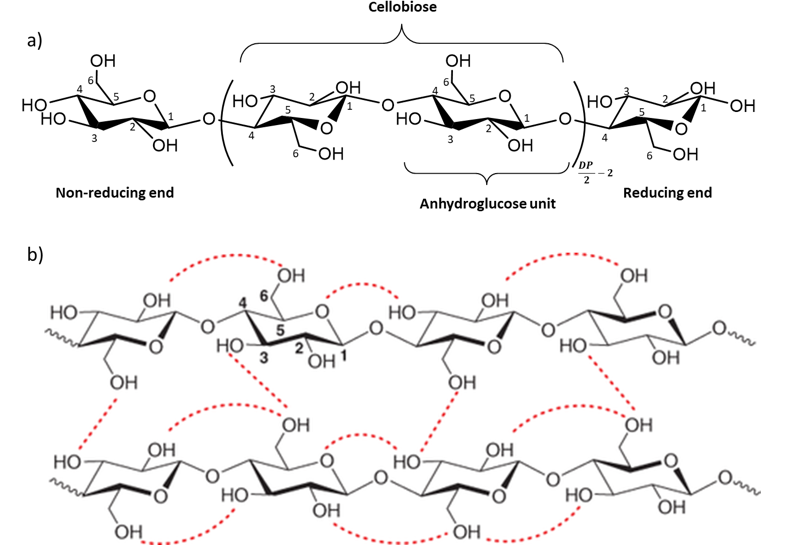
Figure 1 : The molecular structure of cellulose from Klemm et al. (2018) and hydrogen bonding interactions (Kang et al. 2013).
Cellulose fibres and nano-scale cellulose can be extracted from the biomass and used for a wide range of applications. Nanocellulose can be divided into two main types depending on the preparation process and resulting crystalline and morphological characteristics : cellulose nanocrystals (CNCs) and cellulose nanofibrils (CNFs). Their general extraction procedure is illustrated in Figure 2. A third type of nanocellulose exists and is synthesized by bacteria species, mainly of the genera Acetobacter and Agrobacterium. Bacterial cellulose is produced in a very high purity form. Biotechnology processes have been established to produce bacterial cellulose by fermentation (Chawla et al. 2009). Bacterial cellulose is studied for biomedical applications ; however, because of their high cost, only the two main types, CNCs and CNFs, will be discussed.
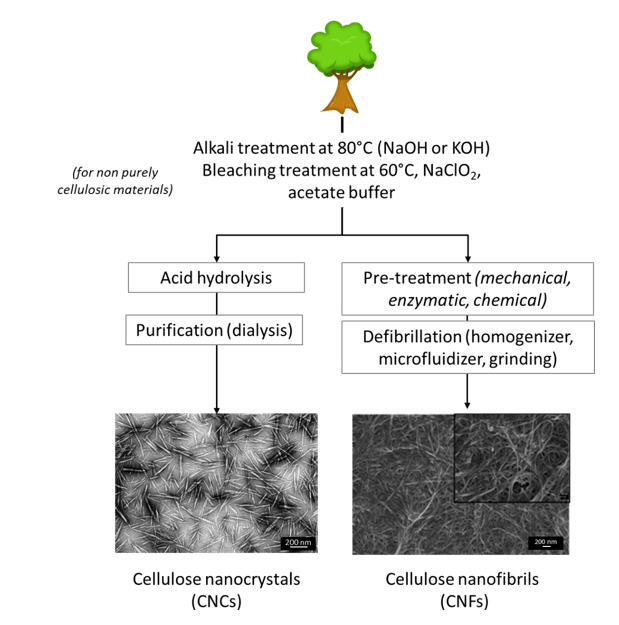
Figure 2 Cellulose nanocrystals and cellulose nanofibrils production. SEM images from (Habibi et al. 2008 ; Lavoine et al. 2014)