On paper, the concept of using naturally occurring carbohydrates in AAT can be considered a revamping of the successful story involving breastfeeding and HMOs. Unsurprisingly, it was implemented as early as the late 70s and was successful to an extent: in vitro and in vivo experiments repeatedly prevented infections in models featuring common pathogens such as E. coli.(Aronson et al., 1979; Sharon, 2006; Sharon et al., 2000) In animals, successful administration of soluble carbohydrates led to the protection of diverse environments: gastrointestinal and urinary tracts, eyes and lungs. Nevertheless, these successes were pushed only to a certain extent, as mono and oligosaccharides showed shortcomings on the prospect of their use for widespread therapy.(Parente et al., 2003; Sharon, 2006; Ukkonen et al., 2000)
Indeed, sugars, by their nature, are not viable therapeutic molecules. The leading problem is that naturally occurring sugars are ‘accounted for’ by human biology, meaning that metabolization machinery does a quick job of degrading them into smaller building blocks for recycling. Indeed, sugars are part of the ‘building-blocks-of-life’, meaning that a cohort of enzymes exists with the sole purpose of assembling, modifying and dismantling carbohydrate structures. Naturally, this would deplete the effective concentration of any sugar, lowering their therapeutic effect and calling for a higher dosage to reach the desired outcome. On a related note, carbohydrate epitopes are found in the glycocalyx and elsewhere, with roles to fill in human biology: communication, immunity or others. It means that overloading the human body with saccharides can have undesired and potentially harmful off-site side effects.
Another issue with sugars is their large polar surface area (PSA). On the one hand, polar molecules are easily dissolved in aqueous solutions, allowing easy administration to epithelial interfaces of infection. On the other hand, the design of therapeutic molecules avoids large PSA values: strongly polar molecules cannot permeate membranes, meaning that some compartments are out of their reach. An excellent example of this issue is biofilms: if the bacterial targets hide behind a lipophilic matrix, polar anti-adhesives are as useless as antibiotics. The PSA of a monosaccharide is already high on the scale of drug design: 120 Å2 for glucose, nearing the 140 Å2 upper limits recommended. It means that oligosaccharides are too polar on this scale.
The last argument separating sugars from drug-like molecules is their low stability : carbohydrates present reactive chemical functions that react with the biological matrix, either facilitated or not by metabolic enzymes. Furthermore, monosaccharides maintain a dynamic equilibrium between different forms (cyclic forms and open chains). It might not be a problem for their biological role, but chemical stability is necessary for a therapeutic entity.
Recapitulating: on the one hand, carbohydrates have proven their anti-adhesive potential both in nature and in the laboratory. On the other hand, they fail to align with what modern medicine considers a ‘viable’ drug. Synthetic organic chemistry has provided a solution to this predicament: glycomimetics. As their name indicates, this relatively new class of therapeutic agents aims to mimic carbohydrates in terms of shape and effect. Their parallel objective is to present an optimized pharmacokinetic profile. The resulting therapeutic molecules, or glycodrugs, boast increased metabolic and chemical stability, specificity for their targets, and the ability to be adjusted and re-designed through organic synthesis to continuously adapt them to new needs.
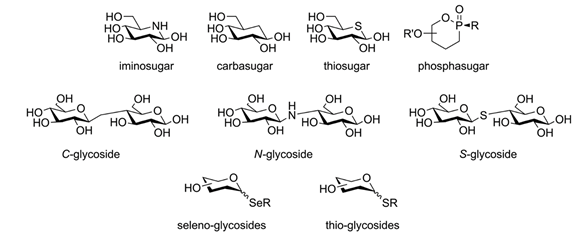
Indeed, glycomimetics are molecules tailored to their target: rather than merely copying the original carbohydrate-mediated interaction, they draw on it and try to perfect it. For example, a representative glycomimetic could be modeled after a monosaccharide but be functionalized with lipophilic moieties that complement the lectin’s binding site to boost affinity and specificity for its target. Under increased lipophilicity, the now moderate polarity of the molecule would grant it access to spaces normally barred for monosaccharides. Finally, the molecule could be synthesized from scratch to replace the ring oxygen with a carbon atom, making it a ‘carbasugar’ as seen in Figure 6. This modification would further reduce the PSA, grant it metabolic stability, and secure the cyclic form from opening. Compared to its monosaccharide equivalent, this hypothetical glycomimetic is already far ahead down the roads of drug-likeness and therapeutic effect.
It’s not necessary to go very far to find a real-life glycomimetic success story: carbohydrate-based oseltamivir/Tamiflu is a widespread antiviral drug that prevents and treats influenza A and B. Oseltamivir was designed to mimic the transition state generated when the viruses’ neuraminidase cleaves the terminal sialic acid of its substrate glycocompounds. Synthetic strategies were applied to boost potency and remove structural weak points detrimental to stability or affinity, starting from a slightly modified monosaccharide. (Ernst et al., 2009; Kim et al., 1997) In addition to installing a hydrophobic moiety to match an apolar pocket of the binding site, the chemical modification also allowed to generate a prodrug derivative, leading to an orally bioavailable glycodrug. Onwards from this early example, the great potential of glycomimetics has sparked a growing number of projects for a range of targets. Some obvious targets for glycomimetics are sugar-metabolizing enzymes: for example, glycodrugs voglibose and miglitol target glycosidases to achieve glycemic control in the context of diabetes. (Campbell et al., 2000; Chen et al., 2006; Ernst et al., 2009) These molecules, an iminosugar and an N-glycoside, are examples of how replacing oxygen atoms with nitrogens can lead to viable glycodrugs. New drugs such as these are always welcome, especially in the case of diabetes: the ever-growing pathology of the modern-day and the eighth leading cause of death in 2012. (WHO, 2016)
A second significant pathology in which glycomimetics have their role to play is cancer: abnormal cancerous cells exhibit unusual modifications in their glycocalyx, opening an avenue for studying and using cancer-related carbohydrates. Indeed, selectins and galectins are lectin families that have shown involvement with cancer and aberrant oligosaccharides.(Natoni et al., 2016; Takenaka et al., 2002) Many glycomimetic antagonists to selectin and galectin are being developed for cancer combination therapy and are currently undergoing clinical trials.(Festuccia et al., 2019; Wdowiak et al., 2018) Apart from targeting these lectin families, glycomimetics have found their way into cancer therapy in other ways. For example, Gemcitabine is a nucleoside analogue that features a fluorinated ribose mimetic used in chemotherapy for decades. (Noble et al., 1997) The list of glycomimetics developed against these and other pathologies is long. Therefore, the synthetic methodologies leading to glycomimetics are ever-growing, as has been recently documented. (Hevey, 2019; Tamburrini et al., 2020)
Similarly, and closer to our interest, glycomimetics have met success as anti-adhesives. Among many successfully drugged targets, we encounter HIV-related DC-SIGN: based on the oligosaccharide epitopes bound by the lectin, new synthetic glycomimetics have been designed and synthesized throughout the years. They can be separated into the two families recognized by DC-SIGN: mannosides mimicking the epitope Man9, and fucosides, mimics of Lewis oligosaccharides. Among the many types of glycomimetics designed, high-affinity monovalent structures were created, mirroring the oligosaccharide assembly, yet replacing each sugar with a glycomimetic counterpart.(Medve et al., 2019; Sattin et al., 2016)
An exciting avenue that synthetic chemistry opens for glycomimetics is covalent inhibition: absent in natural structures, reactive groups can be synthetically added to glycomimetics to tether them to their targets. This strategy applies to carbohydrate-modifying enzymes by taking advantage of their machinery in mechanism-based design. (Ren et al., 2018) Closer to our interest, anti-adhesive covalent compounds that inhibit lectins persistently have shown promising potential to impede the virulence of the corresponding organism. (Wagner et al., 2017)
Nevertheless, the avenue of covalent inhibition entails particular considerations, such as the possibility of unspecific binding and unforeseen side effects. It follows that, for covalent glycomimetic design, ensuring selectivity for the target becomes equally or more important than ensuring high affinity. Incidentally, improving the selectivity and affinity of monovalent ligands is a practical step to take before taking glycomimetics to the next level: multivalency.(Martínez-Ávila et al., 2009; Varga et al., 2014)
Enabled by synthetic chemistry and its tools, the multivalent assembly of glycan ligands has opened the gate to otherwise inaccessible rewards. Ever-increasing numbers of scaffolds and coupling procedures allow straightforward construction of macromolecules bearing repeated units of monovalent ligands. The relevance of multivalent glycocompounds is quite clear: by presenting several copies of the ligand, the monovalent affinities increase to deliver multivalent affinities several orders of magnitude higher. However, this is not new: multivalent glycocompounds aim to emulate nature, which usually handles carbohydrate/lectin interactions with multivalency. Indeed, lectins present many equivalent binding sites simultaneously to compensate for low-affinity monovalent interactions. Furthermore, carbohydrates destined for molecular recognition usually occur in clusters of epitopes yielding the so-called ‘Cluster Glycoside Effect’. This effect, multivalency, and its implications for therapy have been studied and discussed for decades.(Bernardi et al., 2013; Lee et al., 1995; Lundquist et al., 2002; Pieters, 2009) Some important lessons to retain from the use of multivalent glycocompounds relate to their design and their mechanisms of function.
Regarding design, multivalency has infinite possibilities: glycans have been attached to increasingly large frames, and the valency of these structures has exploded accordingly. Some multivalent designs have entirely left behind the idea of drug-likeness to produce therapeutic agents at an entirely different scale: carbohydrates supported by nanoparticles, quantum dots, vesicles, micelles, proteins, polymers, and dendrimers have been successfully implemented as tools or therapeutic agents in various projects.(Ashree et al., 2020; Budhadev et al., 2020; Kim et al., 2005; Prost et al., 2012; Schaeffer et al., 2013; Soria-Martinez et al., 2020; Zubkova et al., 2018) Pushing design to the limit, virus-like structures bearing over a thousand carbohydrates have been generated, bringing the level of mimicry to a new height (Figure 7). (Ribeiro-Viana et al., 2012) Nonetheless, this infinite potential can be regulated by some metrics: the structure’s geometry can be defined by the relative orientation of units and the distance between them. Other factors that have a proven influence are the rigidity of the construction and, naturally, the number of epitopes presented. Particularly in the case of lectins, it has been established that tailoring the multivalent agent to its target (‘lectin-based design’) increases its effectiveness dramatically.(Bernardi et al., 2013; Cecioni et al., 2015; Kane, 2010; Ordanini et al., 2015; Pieters, 2009)
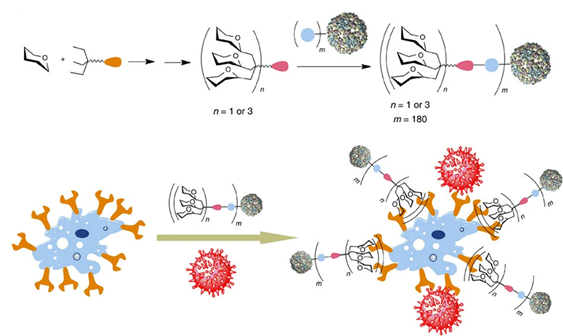
The second lesson to be learned from multivalent glycocompounds relates to their mechanisms of function: more than one effect takes place at the same time when these ligands are confronted with their target. Firstly, it is essential to understand what makes an effective multivalent ligand: comparing it to the monovalent unit is useful to assess its affinity and applicability for practical purposes. This ‘functional’ affinity is called avidity since it results from many equivalent interactions, each with its affinity. Characterizing the efficacy of a multivalent design requires correcting from the multivalent avidity and relating it to a single unit. Comparing this value to the affinity of a monovalent ligand leads to what could be called a relative potency per sugar or epitope. The increase in relative potency observed when sugars are presented multivalently is the true meaning of the ‘multivalent effect’.
With this distinction in mind, it is easier to study the different effects leading to increased affinities and relative potencies, as schematized in Figure 8. The most intuitive effect is chelation, which describes the ability of a molecule to engage two or more binding sites of a target simultaneously. Once a first binding event has established the availability of a multivalent ligand, the affinity of the subsequent interactions is increased compared to the initial binding event. Facilitated binding is one of the drivers of the multivalent effect.
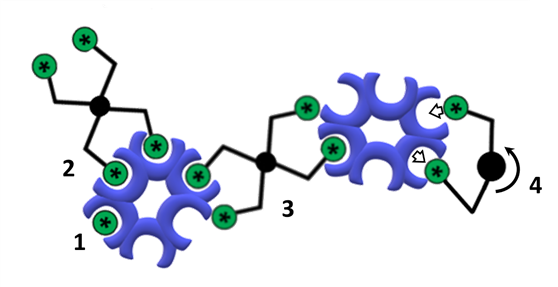
Multivalent design can envision perfectly tailored compounds that fit their lectin targets as a lid fits a pot to push the chelation effect to the limit. Nevertheless, it is a difficult task: any design mistake or fluctuation in the ligand/target dynamics can dramatically affect the affinity measured. Parallel to chelation, a second effect called statistical rebinding describes the increased likelihood of a second interaction happening on the same site where a first binding event has taken place. This effect works synergistically with chelation and drives the chelation effect even further. Importantly, statistical rebinding can also occur in the absence of chelation: a single site may be consecutively engaged by the multiple copies of the ligand presented in a multivalent structure. Therefore, the off-rate of the ligands is reduced, and the affinity is increased. Finally, other effects exist, such as when a compound engages two lectins simultaneously if the steric bulk of the three participants allows it. In this case, the ‘recruitment’ of targets by a multivalent ligand can be called receptor clustering and elicit signalling cascades. (Kiessling et al., 2006) Cross-linking is possible in the case of considerable/long participants with high valencies and can lead to reticulation and even aggregation and precipitation of masses of protein. (Lundquist et al., 2002) This aggregation can be beneficial if the aim is to disable the target, like in AAT. It follows that multivalent compounds can also be designed to encompass various targets at once instead of the ‘lid and pot’ approach. Today, the multivalent glycomimetics design retains a heavy empirical factor, as every target is and behaves differently.
A final word to be said about multivalent glycocompounds is that, although they work well by presenting basic unmodified sugar units, they can benefit from preceding glycomimetic optimization. Indeed, the increased affinity of a monovalent ligand works synergistically: implementing an optimized glycomimetic in a multivalent design can improve its affinity by additional orders of magnitude, as the gain of affinities multiply themselves, rather than adding to each other.(Cecioni et al., 2015; Sattin et al., 2016; Varga et al., 2014)